應用於超寬頻系統之低雜訊放大器與混波器設計
全文
(2) 應用於超寬頻系統之低雜訊放大器與混波器設計. Design of Low Noise Amplifier and Mixer for Ultra-Wideband Application. 研 究 生:林志修. Student:Chih-Hsiou Lin. 指導教授:郭建男. Advisor:Chien-Nan Kuo. 國 立 交 通 大 學 電機資訊學院 電子與光電學程 碩 士 論 文 A Thesis Submitted to Degree Program of Electrical Engineering and Computer Science College of Electrical Engineering and Computer Science National Chiao Tung University In Partial Fulfillment of the Requirements For the Degree of Master of Science In Electronics and Electro-Optical Engineering May 2005 Hsinchu, Taiwan, Republic of China. 中 華 民 國 九十四 年 五 月.
(3) 應用於超寬頻系統之低雜訊放大器與混波器設計. 學生 : 林 志 修. 指導教授 : 郭 建 男 教授. 國立交通大學 電機資訊學院 電子與光電學程 摘要 本篇論文主要是利用標準 0.18µm CMOS 製程設計應用於超寬頻系統前端接 收器之低雜訊放大器和混波器積體電路。在第一顆晶片裡,適用於接收端超寬頻 系統之寬頻放大器被設計與分析。我們利用負回授電阻達到寬頻之輸入輸出組抗 匹配以及自偏壓。實際量測結果顯示此一放大器在 0.1GHz 到 6.6GHz 頻率下有 著最高功率增益(S21) 6.2dB,輸入返回損耗(S11)及輸出返回損耗(S22)小於-10 dB,以及平均雜訊指數 8.1dB,此電路消耗之功率為 16.2mW。 第二顆晶片中,我們利用柴比席夫濾波器來達到寬頻匹配,設計超寬頻系 統接收器第二級之混波器,並在轉導級和混波級中間加入電感來使高頻的增益增 加。實際量測結果顯示此寬頻混波器在 3~8GHz 有最高的轉換增益(Conversion Gain) 7.4dB,輸入返回損耗(S11)小於-4dB,平均 IIP3 為+3.4dBm,最小雜訊指 數 7.1dB。此電路消耗功率為 11.8mW。. i.
(4) Design of Low Noise Amplifier and Mixer for Ultra-Wideband Application. Student: Chih-Hsiou Lin. Advisor: Prof. Chien-Nan Kuo. Degree Program of Electrical Engineering Computer Science National Chiao-Tung University. ABSTRACT This thesis aim is to design an ultra wideband low noise amplifier and mixer in the UWB receiver system using standard 0.18um CMOS process. In first chip, an ultra wideband low noise amplifier is analyzed and designed in UWB system. We employ the negative feedback resistor to achieve input, output broadband matching. In the measured data, we show the maximum power gain is 6.2dB and input matching (S11) and output matching (S22) are less than -10dB from 0.1GHz to 6.6GHz. The average noise figure is 8.1dB. The total power consumption is 16.2mW. In second chip, we design a mixer which is the second stage of the UWB system. In this design, we use the chebyshev filter to achieve 3~8GHz broadband matching. And an inductor adds to between transconductance stage and switching stage which improve the high frequency gain. The measured result shows the highest conversion gain is 7.4dB at 3~8GHz band. Input return loss is less than -4dB. The average iip3 is +3.4dBm. The minimum noise figure is 7.1dB. The total power consumption is 11.8mW. ii.
(5) 誌謝 首先,我要感謝我的指導教授郭建男博士在這二年多來耐心的給與指導和 鼓勵,使我學到許多射頻積體電路方面的知識及設計電路需克服的困難,填補了 以前在工作上似懂非懂的感覺,並感謝一起建立實驗室並共患難的同學們,傅昶 綜是個熱心助人的學長、卓宏達是個電磁學高手、劉上逸、傅敬銘、洪英瑞-常 幫我解決工作站及量測的問題、及個性認真的小馬和喜歡鬥嘴的王柏之與陳仰 鵑,有了大家的努力才有這麼乾淨的讀書環境。還要感謝低功率計畫的指導教授 郭治群教授和成員蔡坤宏-大家都叫他 Dr.岡田,及詹維嘉-書卷獎的常客,從你 們身上獲得其他專業領域的知識。並在課業閒餘,大家一起烤肉,出外踏青,減 輕課業上的壓力。有了以上大家的幫助才能讓我很快的進入狀況並完成論文。 還有大學的專題夥伴兼室友維修,在同一家公司上班,並一起離職到交大 唸書的一段孽緣。並特別感謝球友兼同窗小肥趙在多次寒冷的冬天請吃火鍋的辛 勞及小柯與射手小賊昌的友情贊助。 最後,還要感謝我父母及弟弟志倫在精神上的支持,及遠在澳洲求學和我 常 MSN 的表弟挺毓,讓我能夠安心的在交大完成碩士學位。. 林志修 九十四年 五 月. iii.
(6) CONTENTS ABSTRACT (CHINESE) ...............................................i ABSTRACT (ENGLISH) ..............................................ii ACKNOWLEDGEMENT........................................... iii CONTENTS...................................................................iv. TABLE CAPTIONS ..........................................vi FIGURE CAPTIONS ......................................vii Chapter 1 Introduction..................................................1 1.1. Motivation .................................................................. 1. 1.2 Introduction to Ultra Wideband System.................. 2 1.3 Thesis Organization................................................... 4. Chapter 2 The Fundamentals of Low Noise Amplifier and Mixer........................................................5 2.1. Noise Analysis.......................................................... 5 2.1.1 Source of Noise Type ..................................................................5 2.1.2. Noise Model of MOSFET ..........................................................7. 2.2 Principle of Low Noise Amplifiers............................ 9 2.2.1 Basic Topologies of Low Noise Amplifier .............................9 2.2.2 L-degeneration LNA Noise Analysis .................................... 11. 2.3. Principle of Mixers................................................... 14 2.3.1. Conversion Gain........................................................................15 iv.
(7) 2.3.2. Noise Figure : SSB and DSB ..................................................16. 2.3.3 Isolation .......................................................................................16 2.3.4 Single Balanced and Double Balanced Gilbert Mixer ....17. 2.4 Direct Conversion Receivers................................... 18. Chapter 3 Ultra-wideband Low Noise Amplifier ......21 3.1 Introduction.............................................................. 21 3.2. Principle of the Circuit Design................................ 22 3.2.1 Input Matching principle.........................................................22 3.2.2 Ultra wideband gain flatness analysis .................................24. 3.3 Chip Implementation and Measured Result ......... 27 3.3.1 Microphotograph of Chip........................................................27 3.3.2 Measurement and Simulation Result ....................................28. 3.4 Conclusion ................................................................ 33. Chapter 4 A 3 ~ 8GHz Direct Conversion Broadband Mixer .............................................................34 4.1 Introduction.............................................................. 34 4.2. Principle of the Circuit Design................................ 35 4.2.1 Input Broadband Matching .....................................................35 4.2.2 Inductive-peaking Function ....................................................43 4.2.3 Switching Stage of the 3~8 GHz Direct Conversion Mixer ......................................................................................................................45. 4.3 Chip Implementation and Measured Result ......... 47 4.3.1 Microphotograph of Chip........................................................47 4.3.2 Photograph of On Board Measurement...............................48 4.3.3 On Board PCB Layout Consideration .................................52 4.3.4 Measurement and Simulation Result ....................................53 4.3.5 Discuss and Troubleshooting .................................................57 v.
(8) 4.4 Conclusion ................................................................... 61. Chapter 5 Summary and Future Works ....................62 5.1 Summary ..................................................................... 62 5.2 Future Works .............................................................. 63. REFERENCES.............................................................64 VITA..............................................................................65. TABLE CAPTIONS TABLE 3.1. Summary of measured performance and comparison to other wideband amplifier ………..……………………………………………………..33. TABLE 4.1. Element values for Butterworth Low Pass Filter prototypes ………...39. TABLE 4.2. Element values for Chebyshev Low Pass Filter prototypes..................39. TABLE 4.3. Comparison of simulation and measured performance…………….....57. vi.
(9) FIGURE CAPTIONS Fig. 1.1. Ultra wideband system block diagram........................................................2. Fig. 1.2. Multi-band spectrum allocation…………………………………………...4. Fig. 2.1. Common LNA architectures (a) Resistive termination (b) 1/gm termination (c) Shunt - series feedback (d) Inductive degeneration....……...…..........9. Fig. 2.2. Cascode LNA architecture....……………………...……………..….…...11. Fig. 2.3. Small-signal model for LNA noise calculations……………………..…..11. Fig. 2.4. Single Balanced and Double Balanced Gilbert Mixer……………….......17. Fig. 2.5. (a) Simple direct conversion receiver (b) Direct conversion receiver with Quadrature downconversion…………..………………………….…....19. Fig. 2.6. Self-mixing of (a) LO signal (b) A strong interferer..……….……….......20. Fig. 3.1. Schematic of ultra wideband low noise amplifier……………………......22. Fig. 3.2. Input matching of ultra wideband LNA………………………………….22. Fig. 3.3. Input small signal model and L-deg effect of ultra wideband LNA...…...23. Fig. 3.4. Small signal circuit of ultra wideband low noise amplifier……….….….24. Fig. 3.5. S11 with and without L_deg input matching…………………………….24. Fig. 3.6. Gm with and without series peaking of second stage……………………25. Fig. 3.7. Frequency response of ultra wideband low noise amplifier………...…....25. Fig. 3.8. Microphotograph of UWB LNA………………………………..….....….27. Fig. 3.9. S21 simulation and measured result of UWB LNA…………….…..……29. Fig. 3.10 S11 simulation and measured result of UWB LNA..………..………..….29 Fig. 3.11 S22 simulation and measured result of UWB LNA…………..…....…….30 Fig. 3.12 S12 simulation and measured result of UWB LNA……………….….….30 Fig. 3.13. Noise Figure simulation and measured result of UWB LNA……….…...31. vii.
(10) Fig. 3.14. Linearity at 5GHz of UWB LNA…………………………...………...….31. Fig. 3.15. Linearity measured result versus frequency………………...…….……...32. Fig. 3.16 Group Delay simulation and measured result of UWB LNA……..……...32 Fig. 4.1. Schematic of 3 ~ 8GHz direct conversion broadband mixer..…..…..…...35. Fig. 4.2. The input impedance matching…………………………………………...36. Fig. 4.3. Insertion loss method design procedure…………………………...……..36. Fig. 4.4. Butterworth and chebyshev low pass filter responses (N=3)………...…..38. Fig. 4.5. Ladder circuits for low pass filter prototypes and their element definitions ………….…...………………………………………………………………38. Fig. 4.6. (a) Low pass filter prototype response for ωc=1 (b) Transformation to bandpass response……………………………..….41. Fig. 4.7. Components transformation of low pass filter transfer to bandpass filter (a) Series inductor transformed to series LC (b) Parallel capacitor transformed to shunt LC ….………………………………………………………………………….43. Fig. 4.8. Complete circuit of bandpass filter tansformation…..………………..….43. Fig. 4.9. The inductive peaking function……………………………………..……44. Fig. 4.10. (a) without inductive peaking (b) with inductive peaking…………..……45. Fig. 4.11 Gm curve with and without inductive peaking…………………….……..45 Fig. 4.12 Switching stage of the 3~8GHz direct conversion mixer.………….…….46 Fig. 4.13 Microphotograph of 3~8GHz Direct Conversion Broadband Mixer.….…47 Fig. 4.14. Photograph of on board measurement of broadband mixer………………48. Fig. 4.15. Block diagram of measurement setup…………………………………….49. Fig. 4.16. Photograph of Anaren Balun 30056 and 30057…………………………..49. Fig. 4.17. Return loss of Anaren Balun 30056………………………………………49. viii.
(11) Fig. 4.18. Gain difference of Anaren Balun 30056………………………………….50. Fig. 4.19. Phase difference of Anaren Balun 30056…………………………………50. Fig. 4.20. Return loss of Anaren Balun 30057………………………………………51. Fig. 4.21. Gain difference of Anaren Balun 30057…………………………………51. Fig. 4.22. Phase difference of Anaren Balun 30057………………………………...52. Fig. 4.23. On board PCB layout diagram…………………………………………...53. Fig. 4.24. S11 Simulation and measured result of broadband mixer…………….….54. Fig. 4.25. Conversion gain simulation and measured result of broadband mixer…..54. Fig. 4.26. Linearity measured result at 3GHz of 3 ~ 8GHz broadband mixer……...55. Fig. 4.27. Linearity measured result versus frequency of broadband mixer………..55. Fig. 4.28. Simulation result of Noise Figure of broadband mixer…………………..56. Fig. 4.29. Various width of transmission line of RO4003C………………………...56. Fig. 4.30. S11 Momentum Boardsim with various bondwire value………………...58. Fig. 4.31. Conversion gain Momentum Boardsim with various bondwire value…...58. Fig. 4.32. S11 Momentum Boardsim with two TSMC models………………….….59. Fig. 4.33. Conversion Gain Momentum Boardsim with two TSMC models……….59. Fig. 4.34. Conversion Gain Momentum Boardsim with various parasitic capacitor.... ……………………………………………………………………….…………….…60 Fig. 4.35. Conversion Gain with and without PMOS current source……………….60. Fig. 4.36. Noise figure with and without Momentum Boardsim…………………...61. ix.
(12)
(13) Chapter 1 Introduction 1.1. Motivation Ultra-wideband (UWB) technology has been around since the 1980s[1], but it. has been mainly used for radar-based applications until now, because of the wideband nature of the signal that results in very accurate timing information. However, due to recent developments in high speed switching technology, UWB is becoming more attractive for low cost consumer communications applications. UWB system allows to overlay existing narrowband systems, and result in a much more efficient use of the available spectrum. Therefore, the Federal Communications Commission (FCC) has allocated 7500MHz of spectrum for unlicensed use of ultra-wideband devices (UWB) in the 3.1 to 10.6GHz frequency band. UWB is emerging as a solution for the IEEE 802.15.3a (TG3a) standard. This standard is to provide a specification for a low complexity, low cost, low power consumption, and high data rate wireless connectivity among devices within or entering the personal operating space. The data rate must be high enough (greater than 110Mb/s) to satisfy a set of consumer multimedia industry needs for wireless personal area networks (WPAN) communications. The standard also addresses the quality of service (QoS) capabilities required to support multimedia data types. In order to achieve low power dissipation, low cost and easy to integrate with other device, CMOS (metal oxide semiconductors) offers a good solution for these purposes, although bipolar transistors usually simplify analog circuit design. It offers both active and passive devices. Active devices present cut-off frequencies up to 40GHz and are suitable for RF applications in the wireless communications. However, 1.
(14) the passive devices such as inductors are not working well in RF band because the quality factor is degraded by losses in both the metal and the substrate. In this thesis, we use standard TSMC 0.18µm CMOS process to design an ultra-wideband low noise amplifier and a 3~8 GHz direct convention broadband mixer. Low noise amplifier (LNA) and mixer are the first and second stage in all wireless receiver system. LNA is the first stage in the wireless system that noise requirement is very serious. However the mixer needs high linearity characteristic in the wireless system.. 1.2 Introduction to Ultra Wideband System. Fig. 1.1 Ultra wideband system block diagram Fig. 1.1 is an ultra wideband receiver front end system block diagram. FCC issued in February 2002, allocated 7500MHz of spectrum for unlicensed use of ultra-wideband devices (UWB) in the 3.1 to 10.6 GHz frequency band. It has two possible solutions for the future standard IEEE 802.15.3a (TG3a). The first one is that UWB systems can be designed to use the 7500MHz available UWB spectrum. It means that the signal can be shaped so that its envelope occupies the full spectrum. The other one is that it can be shaped so that it occupies only 500MHz bandwidth, 2.
(15) allowing 15 such signals to cover the entire band. There are, of course, intermediate cases, e.g., five signals of 2.5GHz bandwidth each. We will explain briefly these two methods below.. Impulse Radio: This is single band solution. Information can be encoded in an UWB signal in a variety of methods. The most popular modulation schemes developed to date for UWB are pulse-position modulation (PPM), pulse-amplitude modulation (PAM), and binary phase-shift keying (BPSK). In PPM encoding method which is based on the principle of encoding information with two or more positions in time, referred to the nominal pulse position. A pulse transmitted at the nominal position represents a 0, and a pulse transmitted after the nominal position represents a 1. In PAM encoding method which is based on the principle of encoding information with the amplitude of the impulses. In bi-phase modulation, information is encoded with the polarity of the impulses. The polarity of the impulses is switched to encode a 0 or a 1.. Multi-Band: The multi band system is based on the principle of transmitting different symbols in different bands in a periodic sequence, very similarly to frequency hopping. Each band occupies 500MHz bandwidth, and the hopping rate varies between 16 and 32MHz. The sequences can be used to reduce collisions with other users by using different sequences for different networks. The link margin with respect to 30 and 12ft. The Fig. 1.2 illustrates the division of the UWB spectrum into sub-bands.. 3.
(16) Fig. 1.2 Multi-band spectrum allocation. 1.3 Thesis Organization This thesis discuss about the receiver front-end circuits (low noise amplifier and mixer) design and implementation for the UWB frequency bands. The contents consist of two major topics: “A 0.18µm CMOS ultra-wideband low noise amplifier circuit” and “A 0.18µm CMOS 3~8GHz direct conversion broadband mixer circuit”. In Chapter 2, we will introduce the fundamentals of conventional low noise amplifiers and mixer. And some theoretical MOSFET noise model and noise theory are presented. LNA noise analysis also introduce in this chapter. Furthermore, many important design parameters and direct conversion receiver front end would be presented in this chapter. In Chapter 3, we start to design an ultra-wideband low noise amplifier. The matching method of ultra-wideband amplifiers design is negative resistor feedback plus the inductive degeneration method to enhance bandwidth. And the feedback resistors are the main components to achieve the ultra-wideband matching. In Chapter 4, we design a 3~8 GHz direct convention broadband mixer based on gilbert cell structure. In this work, we will use chebyshev bandpass filter method to satisfy input return loss less than -10dB in our interest band. In Chapter 5, conclusions and future work. Some issues that should be noted for future works on this topic are also summarize. 4.
(17) Chapter 2 The Fundamentals of Low Noise Amplifier and Mixer In this chapter, we will introduce the principles of the low noise amplifier and mixer. Furthermore, this chapter also shows what kinds of parameters and consideration are important in LNA and mixer design. In section 2.1 illustrates the noise sources type and MOSFET noise model [2,3,4]. In section 2.2 shows principle of low noise amplifier and its noise analysis [3,4]. In section 2.3 shows the principle of mixer and some of its important characteristic [5,6]. In section 2.4, we will introduce the advantage and disadvantage of the direct conversion receivers [6].. 2.1 Noise Analysis The LNA is the first stage of the receiver front end. The noise performance is first consideration in design low noise amplifiers. Therefore, the study of noise is very important because it represents a lower limit to the size of electrical signal that can be amplified by a circuit without significant deterioration in signal quality. In this section, the various sources of electronic noise are considered separately. And MOSFET’s noise model will be described here.. 2.1.1 Source of Noise Type In the integrated circuit, there are many kinds of noise sources, such as shot noise, thermal noise and flicker noise (1/f noise). Shot noise is always associated with a direct current flow and is present in diodes, MOS and bipolar transistors. The origin of shot noise can be seen by considering the diode and the carrier concentrations in the device in the forward-bias region. The 5.
(18) passage of each carrier across the junction, which can be modeled as a random event, is dependent on the carrier having sufficient energy and a velocity directed toward the junction. The shot noise current can be represented as i 2 = 2qID∆f , q is the electronic charge ( 1.6 × 10−19 C ), ∆f is the bandwidth in hertz.. Thermal noise is generated from the conventional resistors. It is due to the random thermal motion of the electrons and is unaffected by the presence or absence of direct current, since typical electron drift velocities in a conductor are much less than electron thermal velocities. Since this source of noise is due to the thermal motion of electrons, we expect that it is related to absolute temperature T. The thermal noise can be represented by a series voltage generator v 2 or shunt current generator i 2 . These representations are v 2 = 4kTR∆f , i 2 = 4kT (1/ R)∆f where k is Boltzmann’s. constant. At room temperature: 4kT = 1.66 ×10−20 V − C . Flicker noise is a type of noise found in all active devices, as well as in some discrete passive elements such as carbon resistor. The origins of flicker noise are varied, but it is caused mainly by traps associated with contamination and crystal defects. These traps capture and release carriers in a random fashion and the time constants associated with the process give rise to a noise signal with energy concentrated at low frequencies. Flicker noise, which is always associated with a flow of direct current, displays a spectral density of the form i 2 = K 1( I a / f b )∆f , where ∆f is small bandwidth at frequency f , I is direct current, K1 is constant for a particular device, a is constant in the range 0.5 to 2, b is constant of about unity. It is apparent that flicker noise is most significant at low frequencies, although in devices exhibiting high flicker noise levels, this noise source may dominate the device noise at frequencies well into the megahertz range. 6.
(19) 2.1.2. Noise Model of MOSFET. MOSFET’s noise source mainly comes from gate current noise, drain current noise (channel thermal noise) and flicker noise. Drain current noise id 2 (channel thermal noise) is the dominant noise source of MOS devices. Since MOSFETs are essentially voltage-controlled resistors, they exhibit thermal noise. In the triode region of operation particularly, one would expect noise commensurate with the resistance value. Indeed, detailed theoretical considerations lead to the following expression for the drain current noise of FETs. id2 =4kTγ gd0∆f. (2-1). where gd0 is the drain-source conductance at zero VDS. The parameter g has a value of unity at zero VDS and, in long devices, decreases toward a value of 2/3 in saturation. Note that the drain current noise at zero VDS is precisely that of an ordinary conductance of value gd0. Another kind of thermal noise is gate current noise ig 2 . The fluctuating channel potential couples capacitively into the gate terminal, leading to a noisy gate current. Although this noise is negligible at low frequencies, it can dominate at radio frequencies. The gate current noise may be expressed as ig2 =4kTδ gg∆f gg=. (2-2). ω 2Cgs2 5gd0. where δis the coefficient of gate noise, classically equal to 4/3 for long-channel devices. Equation (2-2) is valid when the device is operated in saturation. The gate noise is partially correlated with the drain noise, with a correlation coefficient given by. 7.
(20) ig × i* d. c≡. ig × id 2. 2. ≈ 0.395j. (2-3). where the value of 0.395j is exact for long-channel devices. The correlation can be treated by expressing the gate noise as the sum of two components, the first of which is fully correlated with the drain noise, and the second of which is uncorrelated with the drain noise. Hence, the gate noise is re-expressed as ig2 =4kTδ gg(1-|c|2 )+4kTδ gg|c|2 ∆f. (2-4). Because of the correlation, special attention must be paid to the reference polarity of the correlated component. The value of c is positive for the polarity. In electronic devices, 1/f noise (flicker noise) arises from a number of different mechanisms, and is most prominent in devices that are sensitive to surface phenomena. Charge trapping phenomena are usually invoked to explain 1/f noise in transistors. Some types of defects and certain impurities can randomly trap and release charge. The trapping times are distributed in a way that can lead to a 1/f noise spectrum in both MOS and bipolar transistors. Larger MOSFETs exhibit less 1/f noise because their larger gate capacitance smooths the fluctuation in the channel charge. Here, if good 1/f noise performance is to be obtained from MOSFETs, the largest practical device sizes must be used (for a given gm). The mean-square 1/f drain noise current is given by in2 =. K gm 2 K ⋅ ⋅ ∆f ≈ ω T 2 ⋅ A ⋅ ∆f 2 f WLCox f. (2-5). where A (=WL) is the area of the gate and K is a device-specific constant. Thus, for a fixed transconductance, a larger gate area and a thinner dielectric reduce this noise term.. 8.
(21) 2.2 Principle of Low Noise Amplifiers In the design of low noise amplifiers, there are several common goals. These include minimizing the noise figure of the amplifier, providing gain with sufficient linearity – typically measured in terms of the third order intercept point, IP3 and providing a stable 50Ω input impedance to terminate an unknown length of transmission line which delivers signal from the antenna to the amplifier. A good input matching is even more critical when a preselect filter precedes the LNA because such filters are often sensitive to the quality of their terminating impedances. The additional constraint of low power consumption which is imposed in portable systems further complicates the design process.. 2.2.1 Basic Topologies of Low Noise Amplifier. Fig 2.1 Common LNA architectures (a) Resistive termination (b) 1/gm termination (c) Shunt - series feedback (d) Inductive degeneration [3] 9.
(22) Here, there are four basic topologies of low noise amplifiers as shown in Fig. 2.1. These techniques provide good stable input impedance matching. The first technique of low noise amplifiers is shown in Fig. 2.1(a). It uses resistive termination of the input port to provide 50Ω impedance. Unfortunately, the use of real resistors in this fashion has a deleterious effect on the amplifier’s noise figure. The second architecture, shown in Fig. 2.1(b), uses the source or emitter of a common gate or common base stage as the input termination. It’s also called 1/gm termination architecture. Assuming matched conditions, yields the following lower bounds on noise factor for the cases of bipolar and CMOS amplifiers: Bipolar:. 3 F= =1.76dB 2. CMOS:. F=1+. γ 5 gm ≥ =2.2dB where α = α 3 gd0. The bipolar representation neglects the effect of base resistance in bipolar devices. In CMOS expressions, γ is the coefficient of channel thermal noise, gm is the device transconductance, and gd0 is the zero bias drain conductance. For long channel devices, γ=2/3, α=1. But in short channel MOS devices, γ can be greater than one, and α can be much less than one. Accordingly, the minimum theoretically achievable noise figures tend to be around 3dB or greater in practice. The third topology is shown in Fig. 2.1(c). This architecture uses resistive shunt and series feedback to set the input and output impedances of the LNA. Amplifiers using shunt-series feedback often have extraordinarily high power dissipation compared to others with similar noise performance. Intuitively, the higher power is partially due to the fact that shunt series amplifiers of this type are naturally broadband, and hence techniques which reduce the power consumption through LC tuning are not applicable. For GPS applications, a broadband front end is not required, 10.
(23) and it is desirable to make use of narrowband techniques to reduce power. The fourth topology is shown in Fig. 2.1(d). It is desirable to have a narrowband RF signal processing, to get rid of out of band blockers. It employs inductive source or emitter degeneration to generate a real term in the input impedance. It offers the possibility of achieving the best noise performance of any architecture.. 2.2.2 L-degeneration LNA Noise Analysis. Fig. 2.2 Cascode LNA architecture. Fig. 2.3 Small-signal model for LNA noise calculations [3] Fig. 2.2 shows the basic cascode LNA architecture. A simple analysis of the input impedance shows that. 11.
(24) Zin=s(Ls+Lg)+. 1 gm1 +( )Ls=ω TLs sCgs Cgs. (at resonance). (2-6). The noise figure of the cascade LNA can be computed by analyzing the circuit shown in Fig. 2.3. In this circuit, RA represents the series resistance of the inductor Lg, Rg is the gate resistance of the NMOS device, id 2 represents the channel thermal noise of the device. ig 2 , c represents the portion of the total gate noise that is correlated with the drain noise. ig 2 , u represents the portion that is uncorrelated with the drain noise. Analysis based on this circuit neglects the contribution of subsequent stages to the amplifier noise figure. Recall that the noise factor for an amplifier is defined as F=. Total_output_noise Total_output_noise_due_to_the_source. (2-7). To evaluate the output noise when the amplifier is driven by a 50Ω source, we first evaluate the transconductance of the input stage. With the output current proportional to the voltage on Cgs, and noting that the input circuit takes the form of a series-resonant network Gm=gmQin=. gm ωT = ω oCgs(Rs+ω TLs) 2ω oRs. (2-8). Qin is the effective Q of the amplifier input circuit. From the equation (2-8), the output noise power density due to the 50Ω source is Sa,src(ω o)=Ssrc(ω o) ⋅ G. 2. m,eff. =. 4kTω T 2. ω o 2Rs(1 +. ω T Ls Rs. (2-9) )2. In a similar way, the output noise power density due to RA and Rg can be expressed as Sa,RA,Rg(ω o)=. 4kT(RA+Rg)ω T 2 ω T Ls 2 ω o 2Rs 2 (1 + ) Rs. (2-10). 12.
(25) Next, the noise power density associated with the correlated portion of the gate noise and drain noise can be expressed as Sa,id,ig,c(ω o)=κ Sa,id(ω o)=. 4kTγκ gd0 ω T Ls 2 (1+ ) Rs. (2-11). where ⎡ δα 2 δα 2 ⎤ κ= | c |2 + ⎢1+ | c | QL ⎥ 5γ 5γ ⎥⎦ ⎢⎣ QL =. α=. ω o(Ls+Lg) Rs. =. 2. (2-12). 1 ω oRsCgs. gm gd0. The last noise term is the contribution of the uncorrelated portion of the gate noise. This contributor has the following power spectral density: Sa,ig,u(ω o)=ξ Sa,id(ω o)=. where. ξ=. 4kTγξ gd0 ω TL s 2 (1+ ) Rs. (2-13). δα 2 (1- | c |2 )(1+Q2L ) 5γ. We observe that all of the noise terms contributed by the first device M1 are proportional to Sa , id (ω o ) , the contribution of the drain noise. Hence, it is convenient to define the contribution of M1 as a whole as Sa,M1(ω o)=χ Sa,id(ω o)=. 4kTγχ gd0 ω T Ls 2 (1+ ) Rs. (2-14). where, after some slight simplification. χ =κ +ξ =1+2|c|QL. δα 2 δα 2 + (1 + Q2L ) 5γ 5γ. (2-15). with (2-14) and (2-15), it is clear that the effect of induced gate noise is to modify the 13.
(26) noise contribution of the device in proportion to χ . It follows directly that F=1+. RA Rg ωo + +γχ gd0Rs( )2 Rs Rs ωT. (2-16). where χ is defined as in (2-15). By factoring out QL from the expression for χ , and noting that gd0QL=. 1 ωT = α ω oRsCgs αω oRs. gm. (2-17). We can re-express F as F=1+. RA Rg γ χ ω o + + ( ) Rs Rs α QL ω T. (2-18). To understand the implications of this new expression for F, we observe that χ includes terms which are constant, proportional to QL, and proportional to Q2L. It follows that (2-18) will contain terms which are proportional to QL as well as inversely proportional to QL. Therefore, a minimum F exists for a particular QL.. 2.3. Principle of Mixers The mixer is an ubiquitous component of wireless systems. An ideal mixer. multiplies the signal at the radio frequency (RF) port with a signal at the local-oscillator (LO) port to create the intermediate-frequency (IF) signal. If the RF and LO signals are sinusoids, it is clear that the IF signal has components at two frequencies. There is a high frequency component at the sum of the RF and LO frequencies, and a low frequency signal at the difference of the RF and LO signals. Therefore, a mixer can effect up conversion or down conversion [5]. In design of RF CMOS mixers is a very challenging task involving trade offs between gain, linearity, noise figure, voltage supply and power consumption.. 14.
(27) 2.3.1 Conversion Gain One important mixer characteristic is conversion gain, which is defined as Conversion Gain=. the desired IF output the value of the RF input. (2-19). The gain of mixers must be carefully defined to avoid confusion. The “voltage conversion gain” of a mixer is defined as the ratio of the rms voltage of the IF signal to the rms voltage of the RF signal. Note that these two signals are centered around two different frequencies. The voltage conversion gain can be measured by applying a sinusoid at ω RF and examining the amplitude of the downconverted component at. ω IF . The “power conversion gain” of a mixer is defined as the IF power delivered to the load divided by the available RF power from the source. If the input impedance and the load impedance of the mixer are both equal to the source impedance, for example, 50Ω, then the voltage conversion gain and power conversion gain of the mixer are equal when expressed in decibels. Conjugate matching at the input of the mixers is necessary in the first downconversion stage of heterodyne receivers that employ image reject filters. The transfer function of these filters is usually characterized for only a standard termination impedance and may exhibit ripples if other impedance levels are used. The load impedance of the mixer, on the other hand, is typically not equal to 50Ω because most passive IF filters have an input impedance of 500 to 1000Ω. In architectures such as homodyne topologies, the load seen by the mixer may be even higher to maximize the voltage gain. From the above observation, we note that the voltage and power conversion gains of a mixer may not be equal in decibels. 15.
(28) 2.3.2 Noise Figure : SSB and DSB Noise Figure is defined as Noise Figure=. signal to noise ratio at RF port signal to noise ratio at IF port. (2-20). In a typical mixer, there are actually two input frequencies that will generate a given intermediate frequency. One is the desired RF signal, and the other is called the image signal. The existence of an image frequency complicates noise figure computations because noise originating in both the desired and image frequencies therefore becomes IF noise, yet there is generally no desired signal at the image frequency. In the usual case where the desired signal exists at only one frequency, the noise figure that one measures is called the single-sideband noise figure (SSB NF); the rarer case, where both the “main” RF and image signals contain useful information, leads to a double-sideband (DSB) noise figure. Clearly, the SSB noise figure will be greater than for the DSB case, since both have the same IF noise but the former has signal power in only a single sideband. Hence, the SSB NF will normally be 3dB higher than the DSB NF.. 2.3.3 Isolation Isolation is one of another parameter in design mixer. It is generally desirable to minimize interaction among the RF, IF, and LO ports. For instance, since the LO signal power is generally quite large compared with that of the RF signal, any LO feedthrough to the IF output might cause problems at subsequent stages in the signal processing chain. This problem is exacerbated if the IF and LO frequencies are similar, so that filtering is ineffective. Even reverse isolation is important in many instances, since poor reverse isolation might permit the strong LO signal to work its way back to 16.
(29) the antenna, where it can radiate and cause interference to other receivers.. 2.3.4 Single Balanced and Double Balanced Gilbert Mixer. Fig. 2.4 Single Balanced and Double Balanced Gilbert Mixer The circuit of single balanced and double balanced Gilbert mixer is shown in Fig. 2.4. The lower stage is operated as a transconductance amplifier which converts RF voltage into a current and then performs a multiplication in the current domain. VLO is chosen large enough so that the transistors alternately switch all of the tail current from one side to the other at the LO frequency. In single balanced mixers, the output consists of sum and difference components, each the result of an odd harmonic of the LO mixing with the RF signal. It makes the linearity worse. So the double balanced mixers exploit symmetry to remove the undesired output LO component through cancellation. Double balanced mixers make the linearity better than single balanced mixers. 17.
(30) In the low voltage applications, the DC current source can be replaced by a parallel LC tank to create a zero headroom AC current source. The resonant frequency of the tank should be chosen to provide rejection of whatever common mode component is most objectionable. If several such components exist, one may use series combinations of parallel LC tanks.. 2.4. Direct Conversion Receivers Fig. 2.5 is a simple direct conversion receiver, where the LO frequency is equal. to the input carrier frequency. The circuit of Fig. 2.5(a) operates properly only with double-sideband AM signals because it overlaps positive and negative parts of the input spectrum. For frequency- and phase-modulated signals, the down-conversion must provide quadrature outputs [Fig. 2.5(b)] so as to avoid loss of information. This is because the two sides of FM or QPSK spectra carry different information and must be separated into quadrature phases in translation to zero frequency. The simplicity of the direct conversion architecture offers two important advantages. First, the problem of image is circumvented because ω IF=0 . As a result, no image filter is required, and the LNA need not drive a 50Ω load. Second, the IF SAW filter and subsequent down-conversion stages are replaced with low pass filters and base-band amplifiers that are amenable to monolithic integration. But this architecture has a DC offset problem. We will discuss this issue in detail below. DC Offsets problem:. Since in a direct conversion topology the downconverted band extends to zero frequency, extraneous offset voltages can corrupt the signal and, more importantly, saturate the following stages. To understand the origin and impact of offsets, consider the receiver shown in Fig. 2.6, where the LPF is followed by an amplifier and an A/D 18.
(31) converter. Let us make two observations. First, the isolation between the LO port and the inputs of the mixer and LNA is not infinite; that is, a finite amount of feedthrough exists from the LO port to points A and B [Fig. 2.6(a)]. Called “ LO leakage,” this effect arises from capacitive and substrate coupling and, if the LO signal is provided externally, bond wire coupling. The leakage signal appearing at the inputs of the LNA and the mixer is now mixed with the LO signal, thus producing a DC component at point C. This phenomenon is called “self-mixing.” A similar effect occurs if a large interferer leaks from the LNA or mixer input to the LO port and is multiplied by itself [Fig. 2.6(b)]. Second, the total gain from the antenna to point X is typically around 80 to 100dB so as to amplify the microvolt input signal to a level that can be digitized by a low cost, low power ADC. Of this gain, typically 25 to 30dB is contributed by the LNA/mixer combination.. Fig. 2.5 (a) Simple direct conversion receiver (b) Direct conversion receiver with Quadrature downconversion 19.
(32) Fig. 2.6 Self-mixing of (a) LO Signal (b) A strong interferer. 20.
(33) Chapter 3 Ultra-wideband Low Noise Amplifier This chapter describes principle of Ultra Wideband Low Noise Amplifier and this chip fabricated by TSMC 0.18μm RF CMOS technology.. 3.1 Introduction Due to data communication capacity increase progressively and portable, the wide bandwidth and low power are the urgent requirements. Therefore, FCC allocated 7500MHz of spectrum for unlicensed use of UWB devices in 3.1 to 10.6GHz frequency band and defined power consumption must less than 250mW. The first stage of the UWB receiver front end is LNA. There are many critical parameters in design ultra wideband amplifier, such as broadband flat gain, good linearity, good noise performance, good group delay, and good input matching from dc to high frequency. Distributed Amplifier (DA) is a popular solution to implement ultra wideband amplifier. DA usually employs transmission line to transfer signal to next stage without loss. Its frequency range can extend to high frequency, but power consumption and die size are very large. Another method is used chebyshev band-pass filter to achieve broadband matching [6]. This method’s don’t consume more power consumption to achieve broadband function. In next chapter, we will use this method to implement a mixer. In this chapter, we employ resistance negative feedback to achieve broadband matching and use skill of pain peaking method to compensate the gain loss at high frequency.. 21.
(34) 3.2. Principle of the Circuit Design In this section, the design of ultra wideband low noise amplifier is presented. Fig.. 3.1 is the schematic of ultra-wideband low noise amplifier. The circuit includes two stage common source amplifiers. M1 is the input transconductance. The resistors, RF1 and RF2 provide input and output matching and self-biasing. The transistor M2 is the second stage transconductance. It provides better isolation and system LNA transconductance gain improve.. Fig. 3.1 Schematic of ultra wideband low noise amplifier. 3.2.1 Input Matching principle. Fig. 3.2 Input matching of ultra wideband LNA 22.
(35) S11. f=10GHz. f=0. without L_deg with L_deg freq (0.0000 Hz to 10.00GHz). Fig. 3.3 Input small signal model and L-deg effect of ultra wideband LNA The LNA input stage is shown as the Fig. 3.2, and Fig. 3.3 is equivalent circuit. The input matching network has combined two matching methods. One is inductive source degeneration matching, and another one is resistive negative feedback. Using this method, the input matching can rather extend to high frequency as Fig. 3.3’s smith chart. If the Cgd is neglected, the input impedance can be expressed as the following Zin1 ≈ Rfm//Zin=. RF1 1 ⎤ ⎡ // ⎢s(Lg1+Ls1)+ω TLs1+ 1-Av1(s) ⎣ sCgs1 ⎦⎥. (3-1). where Rfm is Miller effect impedance of RF1. Av1(s) is the voltage gain of the first stage. In the low frequency, the input impedance is Zin1|ω =0 ≈ Zfm=. RF1 1-Av1(s). (3-2). Depending on equation (3-2), we can observe the resistance is determined by RF1 at the low frequency. At the resonance frequency, Zin1 can express as the following equation. Zin1|ω =ω o ≈. RF1 // ω TLs1 ≈ ω TLs1 1-Av1(s). (3-3). 23.
(36) Depending on equation (3-3), we can observe the Zin1 ≈ ω TLs1 at the resonance frequency. Since these two levels Zin1(ω=0) and Zin1(ω=ω0) give the impedance range as the frequency sweeps, adjusting both levels near 50Ω shall ensure good S11 over the entire frequency band.. 3.2.2 Ultra wideband gain flatness analysis. Fig. 3.4 Small signal circuit of ultra wideband low noise amplifier. -5. S11(dB). -10. -15. without L_deg with L_deg. -20. -25. 0. 2. 4. 6. 8. 10. Freq (GHz). Fig. 3.5 S11 with and without L_deg input matching. 24.
(37) 0.06. Gm(A/V). 0.05. 0.04. 0.03. without series peaking with series peaking. 0.02 0. 2. 4. 6. 8. 10. Freq (GHz). Fig. 3.6 Gm with and without series peaking of second stage. Fig. 3.7 Frequency response of ultra wideband low noise amplifier The Fig. 3.4 is the small signal circuit of ultra wideband amplifier. All the analysis, the Cgd is ignored. The complete voltage gain of ultra wideband LNA can be expressed as Vo Vo1 Vi Zin1 = Gm2 ⋅ (Rd2//RL) ⋅ Gm1 ⋅ (Rd1//Zin2) ⋅ Vo1 Vi Vs Zin1+Rs 1 ≈ ⋅ Gm1 ⋅ Gm2 ⋅ (Rd1//Zin2) ⋅ (Rd2//RL) 2. A v=. 25. (3-4).
(38) where Zin1 ≈ Rfm//Zin=. Zin2=. RF1 1 ⎤ ⎡ // ⎢s(Lg1+Ls1)+ω TLs1+ 1-Av1(s) ⎣ sCgs1 ⎥⎦. RF2+(Rd2//RL) sCgs2 ⋅ ω o2 ⋅ [RF2+(Rd2//RL)] gm2 ⋅ ω o22 ⋅ (Rd2//RL) 1+ + ω o2 ω o2 2 2 s +s +ω o2 s2 +s +ω o2 2 Q2 Q2 2. where Rs is source impedance. Gm1 is the first stage of M1 transconductance. Gm2 is the second stage of M2 transconductance. Zin1 is the input stage impedance. Zin2 is the second stage input impedance. RL is the load impedance. ω o1, ω o2 are the resonance frequency of the first and second stage. The purpose of the first stage of LNA let input matching S11 less than 10dB from dc to 10GHz which is described in section 3.2.1. According to the equation (3-5),. ω o1=1/ (Lg1+Ls1) ⋅ Cgs1 , we can decide the Lg1=0.85nH and Ls1=0.7nH resonance at 10GHz. The Fig. 3.5 shows the condition with and without L_deg and the Gm1’s frequency response is shown in Fig. 3.7(a). Due to Gm1 frequency response is not flatness, we add another stage to compensate high frequency transconductance gain. The Gm2 frequency response is shown as Fig. 3.6. The Lg2=1.2nH and Ls2=0.4nH resonance at 10GHz. The transconductance Gm2 is higher than low frequency at resonance frequency. According to (3-4), the voltage gain is Gm1*Gm2*output resistive. The Fig. 3.7 (c) is the frequency response after the gain compensation. The Gm1, Gm2 can be derived as gm1 1 ⎡⎣s Cgs1(Lg1+Ls1)+sCgs1ω TLs1+1⎤⎦ RF1 gm1 1 = ω o1 s2 +s +ωo12 RF1 Q1. Gm1=. 2. 26. (3-5).
(39) where ω o1=. 1 (Lg1+Ls1) ⋅ Cgs1. Q1=. 1 gm1 ⋅ Ls1 ⋅ ω o1. gm2 1 ⎡⎣s Cgs2(Lg2+Ls2)+sCgs2ω TLs2+1⎤⎦ RF1 gm2 1 = ω o2 s2 +s +ωo22 RF2 Q2. Gm2=. 2. where ω o2=. 1 (Lg2+Ls2) ⋅ Cgs2. Q2 =. 1 gm2 ⋅ Ls2 ⋅ ω o2. 3.3 Chip Implementation and Measured Result 3.3.1 Microphotograph of Chip A microphotograph of the UWB LNA circuit is shown in Figure3.6. The circuit is fabricated in the TSMC 0.18µm CMOS technology. The die area including bonding pads is 0.873 mm by 0.636 mm.. Vdd. Gnd. Vdd. Gnd. RF_in. Gnd Lg1. Lg2 RF out M2. M1 Gnd Ls1. Ls2. Fig. 3.8 Microphotograph of UWB LNA 27. Gnd.
(40) 3.3.2 Measurement and Simulation Result Measurement is conducted by on-wafer RF probing. Measured S-parameters are plotted in Fig. 3.7 to Fig. 3.14, together with simulation results and troubleshooting for comparison. The solid line is the measured data. The circle plot is the simulation result. The square plot is the troubleshooting result. The measured power gain S21 achieves the maximum value of 6.3dB at 100MHz. It is almost matched the simulation result at low frequency. Above 6GHz, the measured power gain start to decrease and deviate from the simulation result. The measured 3dB bandwidth range is from 100MHz to 6.6GHz. Because of the lower gain measured in high frequency, the higher noise figure is measured. The Fig. 3.11 is shown the condition. The difference of measured data and simulation result on the S21 may be due to the lower transconductance. From the equation ω T=gm/Cgs , we can understand the transconductance and cutoff frequency is determined by transistor’s input capacitor. A capacitor (Cp=150fF) is included between the gate and source of the transistor. It is in order to reduce the input transconductance of the transistor for troubleshooting. In the Fig. 3.7, the square plot is the troubleshooting result which is similar to the measured result. The other S-parameters such as S11, S22 and S12 are similar to the simulation result. The Fig. 3.11 is the measured and simulation result of noise figure. The measured data is above the simulation result. The average value of noise figure is 8.1dB. Discrepancy at high frequency may be the degradation of S21 and inaccurate noise model. The linearity analysis is conducted by the two-tone test. The Fig. 3.12 is the linearity measured data at 5GHz. The Fig. 3.13 is the IIP3 measured data at 3~8GHz. The Fig. 3.14 is the group delay simulation and measured data. The simulation result is 67~71ps. The measured result is 40~83ps. The total power of the 28.
(41) UWB LNA is 16.2mW with a power supply 1.8V. The table 3.1 is the summary of measured performance and comparison to other wideband amplifier. 10. S21(dB). 5. 0. -5. Simulation |S21| -10. Wt decrease+variation(Coner=SS) Measured |S21|. -15 0. 2. 4. 6. 8. 10. 12. Freq(GHz) Fig. 3.9 S21 simulation and measured result of UWB LNA. 0. Simulation |S11| Wt decrease+variation(Coner=SS) Measured |S11|. S11(dB). -5. -10. -15. -20. -25 0. 2. 4. 6. 8. 10. Freq(GHz) Fig. 3.10 S11 simulation and measured result of UWB LNA. 29. 12.
(42) -5. S22(dB). -10. -15. -20. Simulation |S22|. -25. Wt decrease+variation(Coner=SS) Measured |S22|. -30 0. 2. 4. 6. 8. 10. 12. Freq(GHz) Fig. 3.11 S22 simulation and measured result of UWB LNA. -10. Simulation |S12| -15. Wt decrease+variation(Coner=SS) Measured |S12|. S12(dB). -20 -25 -30 -35 -40 0. 2. 4. 6. 8. 10. Freq(GHz) Fig. 3.12 S12 simulation and measured result of UWB LNA 30. 12.
(43) 15. Noise Figure(dB). Simulation Noise Figure. Wt decrease+variation(Coner=SS) Measured Noise Figure. 10. 5. 0. 3. 5. 7. 9. 11. 12. Freq(GHz). Output Power(dBm). Fig. 3.13 Noise Figure simulation and measured result of UWB LNA. 10 0 -10 -20 -30 -40 -50 -60 -70 -80 -90 -100. IIP3= -1.5dBm. -50. -40. -30. -20. Input Power(dBm) Fig. 3.14 Linearity at 5GHz of UWB LNA. 31. -10. 0.
(44) 1. IIP3 (dBm). 0. -1. -2. -3 3. 4. 5. 6. 7. 8. Freq (GHz) Fig. 3.15 Linearity measured result versus frequency. 1.2E-10. Group Delay. 1.0E-10 8.0E-11 6.0E-11. Simulation Group Delay. 4.0E-11. Wt decrease+variation(Coner=SS). 2.0E-11. Measured Group Delay. 0.0 0.0. 2.0E9. 4.0E9. 6.0E9. 8.0E9. 1.0E10. 1.2E10. Freq(Hz) Fig. 3.16 Group Delay simulation and measured result of UWB LNA. 32.
(45) TABLE 3.1 Summary of measured performance and comparison to other wideband amplifier BW[GHz]. Gmax[dB]. S11[dB]. NFmin[dB]. IIP3[dBm]. Area[mm2]. P[mW]. Simulation. 0.1-10. 6.7. <-10. 4.1. -3.1. 0.54. 16.2. Measured. 0.1-6.6. 6.2. <-10. 6.5. -1.6. 0.54. 16.2. [7]. 2.9-9.2. 9.3. <-9.9. 4.0. -6.7. 1.1. 9. [8]. 0.6-22. 7.3. <-8. 4.3. N/A. 1.35. 52. [9]. 0.5-4. 6.5. <-7. 5.4. N/A. 0.62. 83.4. [10]. 1.5-7.5. 5.5. <-9.5. 8.5. N/A. 2.86. 216. 3.4 Conclusion In this work, we design an ultra wideband low noise amplifier for the receiver path of UWB system which is used the standard TSMC 0.18µm CMOS process. This circuit uses the feedback resistor and inductive degeneration to achieve the input, output broadband matching. Between two stages, we add an inductor to compensation the high frequency transconductance. The measured result shows that S11, S22 and S12 parameters are similar to simulation result. It shows the L-degeneration method is useful at this work. The measured 3dB frequency range is from 0.1 to 6.6GHz. The measured maximum power gain is 6.2dB. The average IIP3 is -1.6dB. The noise figure minimum is 6.5dB. From Table 3.1, the power consumption of our work is lower than other distributed amplifier [8,9,10] and die size is also small than others.. 33.
(46) Chapter 4 A 3 ~ 8 GHz Direct Conversion Broadband Mixer This chapter describes circuit design principle of a 3~8GHz direct conversion broadband mixer. And this chip fabricated by TSMC 0.18μm RF CMOS technology.. 4.1 Introduction Due to data communication capacity increase progressively, the wide bandwidth and low power are the urgent requirements. Direct conversion receiver (DCR) is most popular architecture in recently years. The frequency of RF signal is the same with the frequency of LO (local oscillator) signal, and down converts to around DC frequency. The homodyne receiver (DCR) offers two important advantages over a heterodyne receiver. First, there are no image problems, so image rejection filter is not required. Second, the SAW filter and subsequent down-conversion stages are replaced with low pass filter and baseband amplifiers that are amenable to monolithic integration. In general, the homodyne receiver just needs small chip area. In this chapter, we employ chebyshev filter to design a 3~8GHz direct conversion mixer. Its advantage has easy to achieve high gain, broadband matching and doesn’t consume extra power but it needs large die size and accurate inductor model. Therefore, this mixer can receive signals from 3~8GHz and down convert to baseband signal directly. The extra current source is used to decrease the current of switch MOS, and increase the lower stage’s transconductance gain. An inductor adds to the inter-stage between the switch and transconductance stage whose the high frequency gain can be improved. 34.
(47) 4.2. Principle of the Circuit Design. Fig. 4.1 Schematic of 3~8GHz direct conversion broadband mixer In this section, the circuit design principle of 3~8GHz direct conversion mixer is presented. This circuit of mixer uses chebyshev filter design to achieve broadband matching. In section 4.2.1 will introduce the broadband matching. Inductor adds to the inter-stage between the switch and transconductance stage then the high frequency gain can be improved. In section 4.2.2 will introduce the inductive peaking function. In order to down covert the RF signal, the mixing stage is needed. In section 4.2.3 will introduce the mixing stage.. 4.2.1 Input Broadband Matching Fig. 4.2 shows the input impedance matching of this circuit. Input matching uses the chebyshev filter design. The filter design technique has two kinds of methods. One 35.
(48) is the image parameter method, and another one is insertion loss method [11]. In this work, we use insertion loss method to design filter. Insertion loss uses network synthesis techniques to design filters with a completely specified frequency response. The design is simplified by beginning with low pass filter prototypes that are normalized in terms of impedance and frequency. Transformations are then applied to convert the prototype designs to the desired frequency range and impedance level. So, the insertion loss method is the most common technique to design filter. The Fig. 4.3 shows the filter design procedure of insertion loss method.. Fig. 4.2 The input impedance matching. Fig. 4.3 Insertion loss method design procedure. 4.2.1.1 Low Pass Filter Prototype In the insertion loss method a filter response is defined by its insertion loss, or power loss ratio, PLR:. PLR=. Power available from source 1 = Power delivered to load 1− | Γ(ω ) |2. 36. (4-1).
(49) where | Γ(ω ) |2 =Γ(ω ) ⋅ Γ * (ω )=Γ(ω ) ⋅ Γ(-ω )= | Γ(-ω ) |2 , so. | Γ(ω ) |2 is an even function of ω we can rewrite | Γ(ω ) |2 as M(ω 2 ) | Γ(ω ) | = M(ω 2 ) + N(ω 2 ) 2. (4-2). where M and N are real polynomials in ω 2 Substituting this form in equation (4-1), we get. PLR=1+. (4-3). M(ω 2 ) N(ω 2 ). Thus, for a filter to be physically realizable its power loss ratio must be of the form in equation (4-3). Notice that specifying the power loss ratio simultaneously constrains the reflection coefficient, Γ(ω ) . We now discuss two practical filter responses below. <1>Butterworth filter: Butterworth response provides the flattest possible passband response for a given filter complexity, or order. For a low pass filter, it is specified by. PLR=1+k 2 (. ω 2N ) ωc. (4-4). where N is the order of the filter, and ω c is the cutoff frequency. The passband extends from ω=0 to ω=ωc. The power loss ratio is 1 + k 2 at the band edge. <2>Chebyshev filter: A chebyshev polynomial is used to specify the insertion loss of an N-order low pass filter as. PLR=1+k 2T 2N (. ω ) ωc. where TN(x)=cos(N ⋅ cos-1x) , x=. (4-5). ω ωc 37.
(50) then a sharper cutoff will result, although the passband response will have ripples of amplitude 1 + k 2 , as shown in Fig. 4.4, since TN(x) oscillates between ±1 for |x| ≤ 1. Thus, k 2 determines the passband ripple level. For large x, TN(x) 1/2(2x)N. Fig. 4.4 Butterworth and Chebyshev Low pass filter responses (N=3) From the power loss ratio equation of butterworth and chebyshev filter, then we could derive the normalized element values of L and C of low pass filter prototype. The element values for the ladder type circuits of Fig. 4.5 and the normalize values of L and C are presented in Table 4.1 and Table 4.2.. Fig. 4.5 Ladder circuits for Low pass filter prototypes and their element definitions 38.
(51) Table 4.1 Element values for Butterworth Low Pass Filter prototypes(g0=1,ωc=1). N (order). g1. g2. g3. 1. 2.0. 1.0. 2. 1.4142. 1.4142. 1.0. 3. 1.0. 2.0. 1.0. g4. 1.0. Table 4.2 Element values for Chebyshev Low Pass Filter prototypes(g0=1,ωc=1). Ripple=0.5dB N (order). g1. g2. g3. 1. 0.6986. 1.0. 2. 1.4029. 0.7071. 1.9841. 3. 1.5963. 1.0967. 1.5963. g4. 1.0. 4.2.1.2 Impedance and Frequency Scaling Impedance scaling: In the prototype design, the source and load resistances are unity (expect for chebyshev filters with even N, which have nonunity load resistance). A source resistance of R0 can be obtained by multiplying the impedances of the prototype design by R0. Then, if we let primes denote impedance scaled quantities, we have the new filter component values given by L'=R0L. (4-6). C R0. (4-7). R's=R0. (4-8). R'L=R0RL. (4-9). C'=. where L, C, and RL are the component values for the original prototype 39.
(52) Frequency scaling for low pass filters: To change the cutoff frequency of a low pass prototype from unity to ωc requires that we scale the frequency dependence of the filter by the factor 1/ωc, which is accomplished by replacing ω by ω/ωc:. ω←. ω ωc. (4-10). then the new power loss ratio will be. P'LR(ω )=PLR(. ω ) ωc. (4-11). where ωc is the new cutoff frequency; cutoff occurs when ω/ωc = 1, or ω=ωc. Therefore, the new element values are determined by applying the substitution of (4-10) to the series reactances, jωLk, and shunt susceptances, jωCk, of the prototype filter. Thus,. jXk=j. ω Lk Lk = jωL'k ⇒ L'k= ωc ωc. (4-12). jBk=j. ω Ck Ck = jωC'k ⇒ C'k= ωc ωc. (4-13). Usually, both impedance and frequency scaling are required, thus the new value is. L'k=. R0Lk. C'k=. (4-14). ωc Ck R0ω c. (4-15). 4.2.1.3 Bandpass Transformation Low pass prototype filter designs can also be transformed to have the bandpass responses illustrated in Fig. 4.6. If ω1 and ω2 denote the edges of the passband, then a bandpass response can be obtained using the following frequency substitution:. 40.
(53) ω←. ω0 ⎛ ω ω0 ⎞ 1 ⎛ ω ω0 ⎞ = ω 2-ω 1 ⎜⎝ ω 0 ω ⎟⎠ ∆ ⎜⎝ ω 0 ω ⎟⎠. where ∆=. (4-16). ω 2-ω 1 ω0. is the fractional bandwidth of the passband. The center frequency, ω0, could be chosen as the arithmetic mean of ω1 and ω2, but the equations are simpler if it is chosen as the geometric mean:. ω 0= ω 1ω 2 Then the transformation of (4-16) maps the bandpass characteristics of Fig. 4.5(b) to the low pass response of Fig. 4.5(a) as follows: When ω =ω 0 ,. 1 ⎛ ω ω0 ⎞ =0 ∆ ⎜⎝ ω 0 ω ⎟⎠. (4-17). When ω =ω 1 ,. 1 ⎛ ω ω 0 ⎞ 1 ⎛ ω 12 -ω 0 2 ⎞ ⎟ =-1 ⎜ - ⎟= ⎜ ∆ ⎝ ω 0 ω ⎠ ∆ ⎝ ω 0 ⋅ω1 ⎠. (4-18). When ω =ω 2 ,. 1 ⎛ ω ω 0 ⎞ 1 ⎛ ω 2 2 -ω 0 2 ⎞ ⎟ =1 ⎜ - ⎟= ⎜ ∆ ⎝ ω0 ω ⎠ ∆ ⎝ ω0 ⋅ω 2 ⎠. (4-19). Fig. 4.6 (a) Low pass filter prototype frequency response for ωc=1 (b) Transformation to bandpass filter frequency response. 41.
(54) The new filter elements are determined by using (4-16) in the expressions for the series reactance and shunt susceptances. Thus,. j ⎛ ω ω0 ⎞ 1 − ⎟ Lk=jωLk'-j jXk= ⎜ ∆ ⎝ ω0 ω ⎠ ω Ck'. (4-20). which shows that a series inductor, Lk , is transformed to a series LC circuit with element values,. Lk'=. Lk ∆ω 0. (4-21). ∆ ω 0 Lk. (4-22). Ck'=. Similarly,. j ⎛ ω ω0 ⎞ 1 jBk= ⎜ - ⎟ Ck=jω Ck'-j ∆ ⎝ ω0 ω ⎠ ω Lk '. (4-23). which shows that a shunt capacitor, Ck , is transformed to a shunt LC circuit with element values,. Lk'=. ∆ ω 0Ck. (4-24). Ck ∆ω 0. (4-25). Ck'=. The low pass filter elements are thus converted to series resonant circuits (low impedance at resonance) in the series arms, and to parallel resonant circuits (high impedance at resonance) in the shunt arms. Notice that both series and parallel resonator elements have a resonant frequency of ω 0. The Fig. 4.7 shows the condition. The Fig. 4.8 is the complete circuit of low pass filter transformed to bandpass filter.. 42.
(55) Fig. 4.7 Components transformation of low pass filter transfer to bandpass filter (a) Series inductor transformed to series LC (b) Parallel capacitor transformed to shunt LC. Fig. 4.8 Complete circuit of bandpass filter tansformation. 4.2.2 Inductive-peaking Function The Fig. 4.9 is the inductive peaking function of single end mixer which help us to understand this function [12,13]. The differential pair mixer is the same as the single end. In this double balanced gilbert mixer, we put an inductor (Lp1) between 43.
(56) the transconductance stage and mixing stage in order to enhance the high frequency gain. The equivalent circuit is shown in Fig. 4.10. The capacitor (Cp) and resistor (R) is the equivalent model that looking into the mixing stage. The capacitor (CL) is the equivalent element which looking into the drain node of transconductance stage. As the Fig. 4.10(a), we can derive Vout=Iin ⋅. R sR(CL +Cp )+1. where ω =. (4-26). -1 ( pole ) R ⋅ (CL+Cp). (4-27). (4-27) is shown the pole location would be decrease the signal at high frequency. As the Fig. 4.10(b) shown, we can derive relationship of current and voltage with inductive peaking function.. Vout=Iin ⋅. R s RCpCLLp1+s CLLp1+sR(Cp+CL)+1 3. 2. (4-28). We can adjust Lp1 to enhance transconductance gain (Gm) at high frequency. The Fig. 4.10 shows the result that with and without inductive peaking.. Fig. 4.9 The inductive peaking function. 44.
(57) Fig. 4.10 (a) without inductive peaking (b) with inductive peaking 0.05. Gm (A/V). 0.04 0.03 0.02. non L-peaking L-peaking. 0.01 0.00 0. 2. 4. 6. 8. 10. Freq (GHz) Fig. 4.11 Gm curve with and without inductive peaking. 4.2.3 Switching Stage of the 3~8 GHz Direct Conversion Mixer The Fig. 4.12 is the mixing stage of this mixer. The main noise source of the mixer comes from the flicker noise. The (4-29) is the noise current representation.. K gm 2 K in= ⋅ ⋅ ∆f ≈ ⋅ ω T 2 ⋅ A ⋅ ∆f 2 f WLCox f 2. (4-29). 45.
(58) From the equation (4-29), we would observe two conditions to reduce noise. One is increase the transistor area size, and another one is decrease the transistor’s gm. The smaller gm means the less current flow of the transistor. The bias current of the transistor set to about 500μA which is around the saturation region. It can improve the noise figure that bias at around the saturation region. The current source is used to enhance the voltage conversion gain and decrease the current flow of the transistor of M3-M6.. Fig. 4.12 Switching stage of the 3~8GHz direct conversion mixer. 46.
(59) 4.3 Chip Implementation and Measured Result 4.3.1 Microphotograph of Chip A microphotograph of the 3~8GHz direct conversion broadband mixer circuit is shown in Fig. 4.13. The circuit is fabricated in the TSMC 0.18µm CMOS technology. The die area including bonding pads is 0.96 mm by 0.985 mm.. Vdd. Gnd. Vb2. Vb1. Vdd. Ls1 Rd1 M3. Ld1. RF+. LO+. M1 LOM2. RF-. M6. Ld2. Rd2 Ls2. IF+ IF-. Gnd. Vdd. Vdd. Fig. 4.13 Microphotograph of 3~8GHz Direct Conversion Broadband Mixer. 47.
(60) 4.3.2 Photograph of On Board Measurement This 3~8Ghz broadband mixer is used by on board probing. The photograph of on board probing is shown as Fig. 4.14. The output stage uses an OP Amplifier (AD9631) as output buffer. The measurement setup is shown Fig. 4.15. The RF port and LO port that we design is differential, so we need to connect a balun to transfer signal. This balun type we choose is Anaren 30056 and 30057 as shown in Fig. 4.16. The measurement characteristic of Anaren 30056 balun is shown from Fig. 4.17 to Fig. 4.19. The Fig. 4.17 is the return loss of balun. The Fig. 4.18 is the gain difference curve. The Fig. 4.19 is the phase difference curve. The Anaren 30057’s characteristic is shown from Fig. 4.20 to Fig. 4.22.. LO Port. IF Port. RF Port Fig. 4.14 Photograph of on board measurement of broadband mixer. 48.
(61) Fig. 4.15 Block diagram of measurement setup. 3. 2. 3. 2. 1. In phase. 1. diff phase. In phase. diff phase. Fig. 4.16 Photograph of Anaren Balun 30056 and 30057. dB(S11, S22, S33). 0 -10 -20 -30. Port 1 Port 2. -40. Port 3. -50 0. 1. 2. 3. 4. 5. Freq (GHz) Fig. 4.17 Return loss of Anaren Balun 30056. 49. 6.
(62) m4 m8 m9 freq=2.000GHz freq=3.000GHz freq=4.000GHz GD=-0.126 GD=0.962 GD=-0.489 10. m4 m8 m9 Gain Diff. 0. -10. Gain Diff S21. -20. S31 -30 0. 2. 4. 6. 8. 10. Freq (Ghz) Fig. 4.18 Gain difference of Anaren Balun 30056. m5 m6 m7 freq=2.000GHz freq=3.000GHz freq=4.000GHz PD=-185.219 PD=180.508 PD=-176.088. 300. m6. Phase Diff. 200 100 0 -100. m5. m7. 2. 4. -200 -300. 0. 6. 8. Freq (GHz) Fig. 4.19 Phase difference of Anaren Balun 30056. 50. 10.
(63) dB(S11, S22, S33). 0 -10 -20 -30. Port 1 Port 2. -40. Port 3. -50 0. 2. 4. 6. 8. 10. 12. Freq (GHz) Fig. 4.20 Return loss of Anaren Balun 30057. m9 m4 m8 freq=4.000GHz freq=6.000GHz freq=8.000GHz GD=-0.473 GD=0.749 GD=-0.507 10. m4 m8 m9 Gain Diff. 0. -10. Gain Diff S21. -20. S31 -30 0. 2. 4. 6. 8. 10. 12. 14. Freq (Ghz) Fig. 4.21 Gain difference of Anaren Balun 30057. 51. 16.
(64) m5 m6 m7 freq=4.000GHzfreq=6.400GHz freq=8.000GH freq=8.000GHz z PD=172.256 PD=-186.251 PD=183.414. 300. Phase Diff. m7. m5. 200 100 0 -100. m6. -200 -300. 0. 2. 4. 6. 8. 10. 12. 14. 16. 18. 20. Freq (GHz) Fig. 4.22 Phase difference of Anaren Balun 30057. 4.3.3 On Board PCB Layout Consideration Because of our broadband mixer design is at high frequency range, we must carefully layout our PCB board. The PCB layout diagram is shown Fig. 4.23. First, the transmission line’s distance between the series inductor and series capacitor (series resonance) can’t be long. In this layout, we just reserve two components’ pad. This is because long distance transmission line would influence our input broadband matching. Second, the parallel inductor and capacitor (parallel resonance) would be layout into the signal path, otherwise, it could causes the loading effect. Then, we put the bypass capacitor 0.1uF near the tank at the bias voltage Vg. It let the ac signal ground. The total length of transmission line between two series resonance can’t exceed 5mm.. 52.
(65) Fig. 4.23 On board PCB layout diagram. 4.3.4 Measurement and Simulation Result Measurement is used on-board probing. The measured S-parameter is plotted in Fig. 4.24, together with simulation results for comparison. The circuit plot is the measured result. The solid line is the simulation result. The triangle plot is the measured result with no balun connection. The low frequency is matched the simulation result, but high frequency is not. The cause is the transmission line performance is not good at high frequency. The board we use is RO4003C. The Fig. 4.28 is the measured result of various transmission line width of RO4003C. In these curves, we can observe the transmission line’s return loss is poor in 3 ~ 6 GHz. The measured maximum conversion gain is 7.4dB at 3-MHz IF band. The Fig. 4.25 is plotted the measured result, together with simulation result for comparison. The circle plot is the measured result. The solid plot is the simulation result. This mixer’s bandwidth is about 3GHz from 2 to 5 GHz. The IIP3 measured result is shown in Fig. 4.26 and Fig. 4.27. The simulation noise figure is shown in Fig. 4.27. The minimum 53.
(66) noise figure is 7.1dB. The total power of this broadband mixer is 11.8mW with a power supply 1.6V. The table 4.3 is the comparison of simulation and measured result. 0. dB(S1,1). -10. -20. Simulation result Measured result Measured result with no Balun On board matching. -30. -40 0. 2. 4. 6. 8. 10. Freq (GHz) Fig. 4.24 S11 Simulation and measured result of broadband mixer. Conversion Gain (dB). 20 15 10 5 0 -5. simulation result measured result. -10 -15 -20 0. 2. 4. 6. 8. 10. Freq (GHz) Fig. 4.25 Conversion gain simulation and measured result of broadband mixer. 54.
(67) Output Power(dBm). 10 0 -10 -20 -30 -40 -50 -60. IIP3=1.23dBm 3GHz two tone test. -70 -80 -23. -18. -13. -8. -3. 2. Input Power(dBm) Fig. 4.26 Linearity measured result at 3GHz of 3~8GHz mixer. IIP3 (dBm). 10. 5. 0. -5. 3. 4. 5. 6. 7. 8. Freq (GHz) Fig. 4.27 Linearity measured result versus frequency of broadband mixer. 55.
(68) Noise Figure (dB). 15. 10. 5 0. 2. 4. 6. 8. 10. Freq (GHz) Fig. 4.28 Simulation result of Noise Figure of broadband mixer. 0. dB(S(1,1)). -10 -20 -30. W=25 mil W=26 mil W=27 mil W=28 mil. -40 -50 0. 2. 4. 6. W. 8. Freq (GHz) Fig. 4.29 Various width of transmission line of RO4003C. 56. 10.
數據

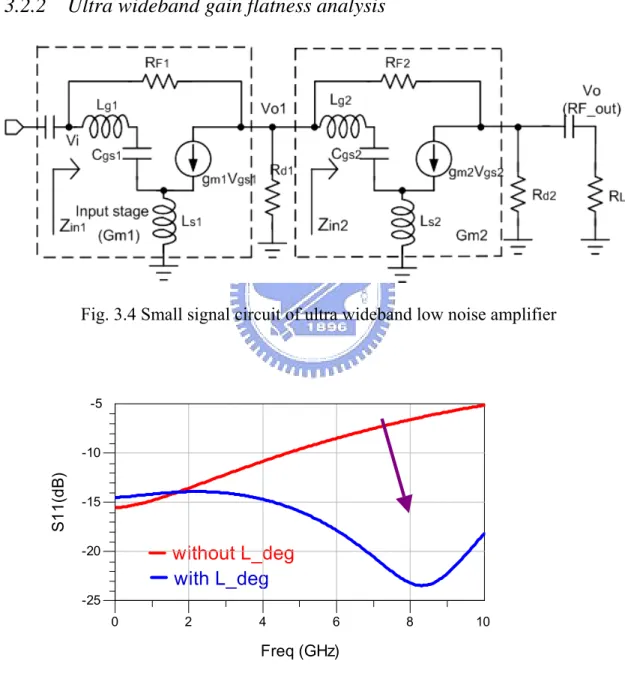
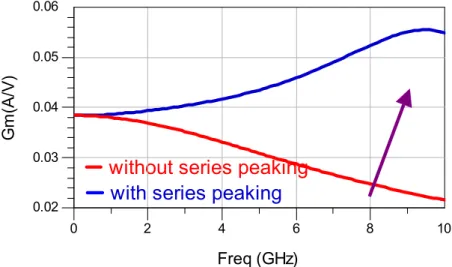
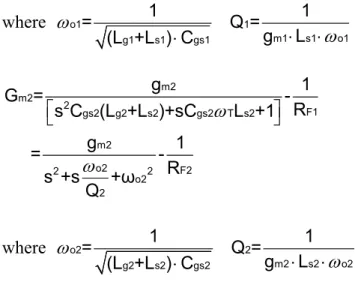
相關文件
• Atomic, molecular, and optical systems provide powerful platforms to explore topological physics. • Ultracold gases are good for exploring many-particle and
If growing cities in Asia and Africa can provide clean, safe housing, the future of the people moving there should be a very good one... What is the main idea of the
classroom management skills and the application of good teaching practices in both reading and
○ Value function: how good is each state and/or action. ○ Policy: agent’s
= special contextual bandit + verified instances as rewards.. Good Properties of Greedy. Case 1: positive
◦ Value function: how good is each state and/or action.. ◦ Policy: agent’s
首先,在前言對於為什麼要進行此項研究,動機為何?製程的選擇是基於
provides a high-speed network environment. Using HP Proliant DL380 server with Gigabit Ethernet bandwidth as test machines, SRB has very good performance in